Fundus photography, fundus fluorescein angiography, and optical coherence tomography of healthy cynomolgus monkey, New Zealand rabbit, Sprague Dawley rat, and BALB/c mouse retinas
Highlight box
Key findings
• Monkey is a good model to study changes in retinal structure associated with fundus disease, rabbits are not suitable for studies on retinal vessel diseases and optic nerve diseases, and rats and mice are good models for retinal vascular diseases.
What is known and what is new?
• Numerous reports have examined normal parameters for blood, heart, brain, liver, kidney, and other large organs among common experimental model animals, but few have examined eye structure and function in different models for ophthalmology research. In this study, we preformed fundus photography, FFA, and OCT examinations in healthy cynomolgus monkeys, New Zealand rabbits, Sprague Dawley rats, and BALB/c mice to provide precise baseline parameters for future studies.
What is the implication, and what should change now?
• Our study will help guide the choice of model and measurement technology and reduce the number of experimental animals required.
Introduction
The choice of experimental animal and measurement technology is critical for the applicability and translation of basic medical research to human diseases. Qualitative and quantitative changes in anatomical structure and physiological function are the basis of disease research, so accurate descriptions of baseline properties are essential. Numerous reports have examined normal parameters for blood, heart, brain, liver, kidney, and other large organs among common experimental model animals (1-5), but few have examined eye structure and function in different models for ophthalmology research. A complete set of anatomic and physiological parameters for specific experimental animals will be invaluable for choosing the optimal model, preparation technique, and measurement technology. Further, high-precision measurements can increase statistical power and broaden the scope of analysis to earlier or less severe conditions. Fundus fluorescein angiography (FFA) is a mainstay method for evaluating changes in retinal blood flow (6) that uses sodium fluorescein as a contrast agent to reveal vessel pattern and integrity. Continuous image capture by fundus camera allows for dynamic observation of retinal vessels during disease development for diagnosis, prognosis, and treatment evaluation. Optical coherence tomography (OCT) is a newer optical imaging technique that has shown rapid development these years (7). It is based on the principle of weak coherent light interferometry, in which differential light scatter and reflection among individual tissue types can be used to construct two-dimensional and three-dimensional images of complex organs. It is widely applied in ophthalmology for the non-contact and non-invasive analysis of living eye microstructure, and has yielded high-resolution images of morphological structures in both the anterior and posterior segments of the eye. Therefore, OCT has important applications in the diagnosis and research of intraocular diseases, especially retinal diseases.
In this study, we preformed fundus photography (FP), FFA, and OCT examinations in healthy cynomolgus monkeys, New Zealand rabbits, Sprague Dawley (SD) rats, and BALB/c mice to provide precise baseline parameters for future studies. We also suggest the best models and inappropriate models for specific applications. We present this article in accordance with the ARRIVE reporting checklist (available at https://aes.amegroups.com/article/view/10.21037/aes-22-54/rc).
Methods
Animals
All animals in this study were adults. There were three conventional (CV) male cynomolgus monkeys (Guangzhou Yuanyuan Animal Breeding Co. Ltd., Guangdong, China) weighing ~4.0–5.0 kg, six CV male New Zealand rabbits (Huadong Xinhua Experimental Animal Farm, Guangdong, China) weighing ~1.5–2.0 kg, ten specific pathogen free (SPF) male SD rats weighing ~180–220 g, and ten SPF male BALB/c mice (Jinan Pengyue Experimental Animal Breeding Co. Ltd., Shandong, China) weighing 18–22 g were used in these studies. All animals were housed according to national standard GB 14925-2010 of the People’s Republic of China. Monkeys and rabbits were housed under the following conditions: temperature 25±1 ℃, humidity 60%±5%, air purification Level 8, noise ≤60 dB, ammonia concentration ≤14 mg/m3, light/dark cycle 12 h/12 h. Rats and mice were housed in a barrier environment under the same temperature, humidity, air purification level, noise level, ammonia concentration, and light cycle. All animals were given free access to water and food and all were acclimated for a week before experiments. Both eyes were then examined using FP, FFA, and OCT. All animal procedures were performed in accordance with the Association for Research in Vision and Ophthalmology (ARVO) Statement for the Use of Animals in Ophthalmic and Vision Research and approved by the Institutional Animal Ethics Committee of Zhongshan Ophthalmic Center, Sun Yat-sen University (Animal Welfare Assurance No. 2016-120). Monkeys in this research would send back to the farm for semi-free range after experiments. Mice, rats and rabbits would proceed euthanasia after the research. They would be injected with over dose of potassium chloride solution after successful anesthesia.
Anesthesia
Cynomolgus monkeys and New Zealand rabbits were anesthetized by intramuscular injection of 3% sodium pentobarbital (0.5 mL/kg, Sigma-Aldrich, Darmstadt, Germany) and 0.1 mL/kg xylazine hydrochloride (Su-Mian-Xin II, Shengda Animal Drug Company, Dunhua City, China), SD rats by intraperitoneal injection of 3% sodium pentobarbital (1 mL/kg), and BALB/c mice by intraperitoneal injection of 0.3% sodium pentobarbital (0.1 mL/mg). After successful induction of general anesthesia, tropicamide eye drops (Mydrin, Alcon, Fort Worth, Texas, USA) were administrated to dilate pupils. During examination, animals were kept warm using an electric blanket and the corneas kept moist by application of artificial tears (from the pharmacy of Zhongshan Ophthalmic Center, Guangzhou, China).
FP and fundus fluorescence angiography
Under general anesthesia, fundus photographs were acquired centered on the macula of cynomolgus monkeys or the optic disc of New Zealand rabbits, SD rats, and BALB/c mice using a fundus camera (monkeys and rabbits: TCR-50DX, Topcon, Tokyo, Japan; rats and mice: PHOENIX Micron IV, Phoenix Research Laboratories Inc., Pleasanton, CA 94588, USA). After baseline FP, cynomolgus monkeys were injected with 1 mL of 20% fluorescein sodium (Guangzhou Baiyunshan Mingxing Pharmaceutical Company, Guangzhou, China) in 5 s via the small saphenous vein and New Zealand rabbits with 1 mL of 10% fluorescein sodium solution via the ear vein. Early-phase photographs were acquired during the first 2 min post-injection and late-phase photographs over 8–10 min post-injection. Rats were injected with 0.1 mL of 10% fluorescein sodium and mice with 0.1 mL of 2.5% fluorescein sodium (same supplier) into the peritoneal cavity. Early phase photographs were taken during the first 2 min post-injection and late-phase photographs 6–10 min post-injection.
OCT
We used the same OCT system for all animals (SpectralisOCT, Heidelberg Engineering, Heidelberg, Germany). For retinal thickness measures, cynomolgus monkeys were scanned horizontally and vertically at the macular fovea and the average was recorded. New Zealand rabbits were scanned over a 7 mm × 7 mm area centered on and below the optic disc while SD rats and BALB/c mice were scanned over a 7 mm × 7 mm area including the center of the optic disc. For all animals, retinal thickness was defined as the distance from the internal limiting membrane (ILM) and retinal pigment epithelium (RPE) to the Bruch membrane. For retinal nerve fiber thickness analysis, all animals were scanned in a round area centered on the optic disc.
Statistical analyses
Parameters from right and left eyes were compared by paired-sample t tests while other parameters were compared by independent samples Student’s t-tests. Multiple means were compared by analysis of variance (ANOVA) with post hoc Bonferroni tests for pair-wise comparisons. All statistical analyses were performed using SPSS 20.0 software (IBM Corporation, Armonk, New York, USA). Data are expressed as mean ± SD. Statistical significance was set at P<0.05 (two-tailed) for all tests.
Results
FP
On fundus photographs of cynomolgus monkey (Figure 1A), there were a clear optic nerve head, macula, and retinal vessels, like human retina. The optic nerve head of cynomolgus monkey was oval (vertical in the long axis) and orange-red. Its boundary was clear and the cup to disc (C/D) ratio was about 0.2. Retinal arteries were bright red, veins were dark red, and the mean ratio of artery to vein diameter (A/V) was about 2:3. The macula was located on the temporal side of the optic disc, 1.5 vertical optic disc diameters (1.5 PD) from the optic disc. It was taupe in color and free of vessels. The healthy retina was flat and even, pigmentation was symmetrical in all directions.
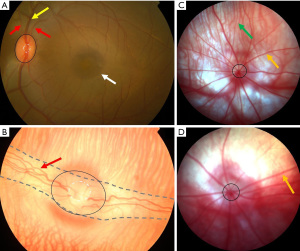
Figure 1B presents FPs of New Zealand rabbit retina showing the optic nerve head, retinal vessels, and choroid. The optic nerve head was oval (horizontal in the long axis) and orange-red. The boundary was unclear and the C/D ratio was about 0.4–0.5. Ivory-colored myelinated nerve fibers and retinal vessels were observed passing through the optic disc. Compared to retinal veins, retinal arteries were brighter and thinner (A/V of about 2:3). Healthy New Zealand rabbit retinas were flat and even, no macular-like structure and pigment were observed, but they have a structure called area centralis (cone rich region) similar to the human macula. The choroid was visible beneath the retina but the macula could not be distinguished.
Fundus photographs of SD rats revealed a clear optic nerve head, retinal vessels, choroid (Figure 1C). The optic nerve head was round and light yellow. The boundary was clear but with no obvious optic cup structure. No macula was detected, too. Retinal vessels were radially distributed. Healthy retinas were flat and even, without pigmentation. Circuitous choroid vessels were observed beneath the retina.
The BALB/c mouse retina (Figure 1D) appeared similar to the SD rat retina on fundus photographs. The optic nerve head was quasi-circular, light yellow, and exhibited no clear boundary or optic cup structure. No macula was observed. Retinal vessels were radially distributed. Healthy retinas of BALB/c mice were flat and even, with no pigment. Choroid was observed beneath the retina but the fine structure was unclear.
New Zealand rabbits, SD rats, and BALB/c mice are albino strains. In New Zealand rabbits and SD rats, the individual structures and distribution of choroid vessels were clearly visible beneath the retina. However, the choroid of BALB/c mice was indistinct.
Fundus fluorescence angiography
After passing through the optic disc, the two retinal arteries of cynomolgus monkey bifurcated to form 4 arteries supplying the superior temporal, inferior temporal, superior nasal, and inferior nasal quadrants of the retina. The retinal veins ran in parallel with the arteries and converged first into upper and lower veins then a central vein before leaving the retina. The mean time from injection of fluorescein sodium into the monkey small saphenous vein to appearance in the retina was 10.7±0.6 s. During the early phase of angiography (0–2 min post-injection), individual choroid capillaries filled with fluorescent dye and progressively became indistinguishable. Then the primary and secondary arteries filled, followed by the secondary and primary veins. The optic disc was also stained. During the late phase (8–10 min), fluorescence in retinal vessels faded and the optic disc appeared as a halo due to residual staining. There were no vessels at the macula, so this area remained fluorescence-free throughout image acquisition (Figure 2A).
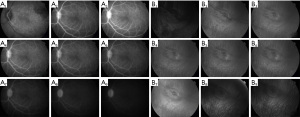
The two primary retinal arteries of New Zealand rabbits distributed to the nasal and temporal sides of the optic disc, emitting smaller branches along the way. Two large veins ran in parallel with the arteries, then passed through the optic disc. Circulation time from injection of fluorescein sodium into the ear vein to the retina was 8.4±2.1 s. During the early phase, choroid became visible, followed by retinal arteries and veins in succession. During the late phase (6–10 min post-injection), vascular fluorescence faded, and optic disc fluorescence eventually disappeared. Individual choroid vessels filled with fluorescent tracer during the early phase and eventually became indistinguishable. The fluorescence faded more rapidly from choroid than from retinal vessels (Figure 2B).
SD rat retinal vessels emerged from the optic disc, travelling straight and then radially. An average of 13.6±2.3 vessels was observed in each rat retina. During angiography, retinal vessels became fluorescent rapidly and faded quickly. Due to the absence of pigment, the background fluorescence from the choroid was strong and interfered with the resolution of retinal angiography. However, vessels of the choroid may have filled and emptied rapidly compared to the acquisition rate, such that much of this process was missed (Figure 3A).
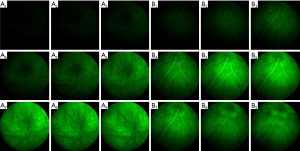
Retinal vessels of BALB/c mice emerged from the optic disc and formed an average of 10.7±1.3 branches that distributed radially. During the early phase of FFA, retinal vessels gradually filled with fluorescein, and individual capillaries could be distinguished. During the late phase, large branches emptied but the vascular walls remained stained. Like SD rats, background fluorescence from the choroid was strong (Figure 3B).
OCT
The structure of the cynomolgus monkey retina looks like the human retina on OCT images. These images distinguished 11 retinal layers, the retinal nerve fiber layer (RNFL), ganglion cell layer (GCL), inner plexiform layer (IPL), inner nuclear layer (INL), outer plexiform layer (OPL), outer nuclear layer (ONL), external limiting membrane (ELM), myoid zone, ellipsoid zone, photoreceptor outer segment (OS), RPE and Bruch’s membrane (RPE/BM) (8-10), in addition to the underlying choroid (Figure 4A). Macular foveal thickness did not differ between right eyes and left eyes (207.17±14.77 vs. 209.43±5.26 µm, P>0.05). Alternatively, RNFL thickness differed significantly among the 4 quadrants (Table 1) (P=0.001), with significant pair-wise differences between temporal and inferior (P=0.008), superior and nasal (P=0.005), and inferior and nasal (P=0.001) quadrants.
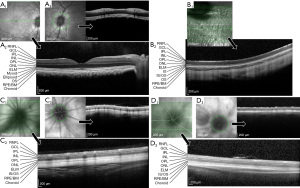
Table 1
Area | Temporal | Superior | Nasal | Inferior | Whole area |
---|---|---|---|---|---|
Monkey | |||||
RNFL | 82.93±4.62 | 124.51±20.49 | 56.07±4.62 | 145.56±22.98 | 113.19±16.27 |
Rabbit | |||||
Retina | |||||
3–6 mm* | 304.6±65.69 | 163.2±30.04 | 308.1±35.18 | 228.9±44.04 | 251.20±34.78 |
Rat | |||||
Retina | |||||
1–3 mm* | 242.11±11.55 | 234.22±11.97 | 245.94±11.12 | 258.89±21.73 | 245.29±12.19 |
3–6 mm* | 229.72±8.96 | 224.94±8.93 | 226.89±7.73 | 234.44±15.27 | 229.00±9.46 |
RNFL | 45.47±2.45 | 43.56±2.66 | 47.47±2.45 | 51.98±4.00 | 47.33±2.58 |
Mouse | |||||
Retina | |||||
1–2.22 mm* | 215.47±12.82 | 218.47±13.46 | 214.44±16.72 | 214.22±11.86 | 215.65±12.61 |
2.22–3.45 mm* | 227.22±8.71 | 233.33±19.88 | 229.92±17.89 | 234.47±17.68 | 231.24±13.74 |
RNFL | 39.18±8.08 | 37.56±7.06 | 39.76±7.65 | 37.91±8.00 | 38.32±7.34 |
Data are shown as mean ± SD. *, 1–3 mm: in an annular region centered on the optic disc with a diameter of 1–3 mm; 3–6 mm: in an annular region centered on the optic disc with a diameter of 3–6 mm; 1–2.22 mm: in an annular region centered on the optic disc with a diameter of 1–2.22 mm; 2.22–3.45 mm: in an annular region centered on the optic disc with a diameter of 2.22–3.45 mm. RNFL, retinal nerve fiber layer; SD, standard deviation.
Outside the optic disc, 11 layers were still observed in New Zealand rabbits’ retina, including (RNFL, GCL, IPL, INL, OPL, ONL, incomplete ELM, photoreceptor inner segment (IS), junction of outer and inner segment, photoreceptor OS, and RPE/BM) (Figure 4B) (11-13). The average thickness of New Zealand rabbit retina was 251.20±34.78 µm. Retinal thickness values within a 3–6 mm annular region centered on the optic disc are presented in Table 1. There was no significant difference between temporal and nasal retina thickness (P>0.05). Due to the large optic disc of New Zealand rabbits, the annular region within 1–3 mm and RNFL thickness could not be measured.
Due to the multitude of retinal vessels in SD rats, many vascular shadows were observed by OCT, which partly obscured the RNFL. Nonetheless, 9 retinal layers could still be distinguished [RNFL, GCL, IPL, INL, OPL, ONL, ELM, junction of outer and inner segment (IS/OS), and RPE/BM] (Figure 4C) (14,15). Retina and RNFL thickness values across the retina are summarized in Table 1. Statistically significant differences in rat retinal thickness were found between annular regions of diameter 1–3 and 3–6 mm (P=0.000), and between superior and inferior quadrants within the annular region of diameter 1–3 mm (P=0.01). Mean RNFL thickness also differed significantly among the 4 quadrants (P=0.000) and the inferior quadrant differed significantly from all three other quadrants (temporal P=0.000, superior P=0.000, nasal P=0.022).
Like SD rats, numerous vascular shadows were seen on OCT images of BALB/c mouse retina. Nonetheless, 9 layers could be resolved (RNFL, GCL, IPL, INL, OPL, ONL, ELM, IS/OS, and RPE/BM) (Figure 4D) (16). The thickness distribution in different areas of retina and RNFL are summarized in Table 1. There was a statistically significant difference in retinal thickness between the annular region 1–2.22 mm from the optic disk and that 2.22–3.45 mm from the optic disk (P=0.000), but no significant differences among quadrants within these annular regions (P>0.05). Mean RNFL thickness did not differ among quadrants (P>0.05).
Discussion
Most retinal structures of cynomolgus monkey were anatomically similar to the corresponding human structures as revealed by FP, FFA, and OCT. A notable exception was the monkey optic disc shape, which was oval with long-axis in the vertical direction whilst the human structure in more symmetrically round. The circulation time from the small saphenous vein to retina was also approximately equal to that in human. The pattern of RNFL thickness variation in different directions from the optic disc was also similar to human, with greater thickness in superior and inferior quadrants compared to temporal and nasal quadrants. Therefore, the cynomolgus monkey is a very good experimental model to study structural changes associated with fundus diseases.
The large eyeball of the New Zealand rabbit permits easy experimental manipulation, measurement, and dissection, and so is widely used in ophthalmologic research. However, FP and FFA revealed two notable differences compared to human retina, a larger optic disc and myelinated retinal nerve fibers (17,18). There were also substantial differences in the growth pattern of retinal vessels compared to the human retina. Retinal vessels of New Zealand rabbits distributed in a small area of temporal and nasal optic disc, while a larger area was supplied by the choroid. The temporal and nasal areas of retina were significantly thicker than superior and inferior areas due to the myelinated fiber distribution as revealed by FP, which is also distinct from human retina. The inferior region of the rabbit retina was also thicker than the superior area. Thus, to track changes in retinal thickness among individual animals, it is critical that measurements are taken at the same location. In fact, there is substantial variation in retinal thickness among studies, which may be explained by inconsistencies in measurement location or different kinds of rabbits (11-13). We could not analyze RNFL thickness in rabbits because the large optic disc obscured the RNFL on OCT images. In conclusion, New Zealand rabbits are not suitable for research on optic nerve diseases. But rabbit is well recommended by Food and Drug Administration (FDA) to evaluate the safety and tox of the ocular compounds. In addition, in the vision filed, retinopathy model and DL-AAA RNV models of rabbit have been used to test the efficacy to various retinal drugs.
Compared to monkeys and rabbits, the retinal vessels of SD rats and BALB/c mice were widely distributed and clear. However, the smaller rat and mouse eyeballs require precise laboratory techniques and high-resolution measurements. Retinal thickness differed according to distance from the optic disc on OCT images. Therefore, to compare retinal changes at different time points within the same animal, it is critical to choose the same retinal location. In mice, RNFL thickness also differed by orientation, which may yield artifactual differences if the measurement site is inconsistent. In rats, inferior RNFL and inferior retina were thicker than the superior RNFL and superior retina, respectively, possibly due to the greater number of retinal vessels. However, due to the small eye and high vessel density, RNFL thickness is inherently difficult to measure accurately in rats and mice. Further, neural fibers and vessels were highly reflective, which interfered with OCT resolution. Therefore, we suggest that the “ganglion cell complex (GCC)” (19,20) including the RNFL, GCL, and IPL be used as a metric for retinal ganglion cell changes in rats and mice rather than the RNFL. The GCC includes all major compartments of retinal ganglion cells, while the RNFL is mainly composed of axons, the GCL of somata, and the IPL of dendrites. Therefore, GCC is a superior metric for overall condition (but not for specific changes in individual compartments). Measurement of the GCC may also reduce errors caused by retinal blood vessel changes.
The vascular structures of the retina can also be revealed by stretched preparations, and results do not appear to differ from FFA findings (21). However, FFA images are not influenced by operative manipulations or post-mortem changes, and can also reveal changes in retinal vessel pattern and flow over time in the same preparation. Thus, FFA is recommended for studies on retinal vessel diseases. In this research, the injected concentrations and volumes of contrast agent were obtained from literature review (22-26) and our experimental experience. At high concentrations, signals rose faster and higher to reveal the overall vascular structure quickly and in detail. However, lower concentrations offered more time for observation during the early phase.
In the three albino strains, the choroid was clearly seen on FP and signals from choroid were high on FFA, due to the absence of pigment, which obscured the resolution and detection of retinal vessels. Therefore, albino strains are not suitable for studies on retinal vessel diseases by only FFA, but by indocyanine green angiography (ICGA). On the other hand, due to the lack of pigment, albino strains are good models to study choroid diseases.
Conclusions
We examined the healthy retinas of cynomolgus monkey, New Zealand rabbit, SD rat, and BALB/c mouse by FP, FFA, and OCT to identify models most appropriate for specific experimental applications in ophthalmology. The structure of the cynomolgus monkey fundus was closest to human, and so cynomolgus monkey is the best model for examination of fundus lesions, although the cost is also highest. New Zealand rabbits have large eye balls, so experimental manipulations and measurements are easier than in other small mammal models such as mice and rats. However, fundus structure is much different from that of human, so this is an unsuitable model for fundus disease research. The retinal vessels of SD rats and BALB/c mice were clear, and retinal structure were similar to human. While the smaller eyeballs increase the difficulty of experimental manipulation and measurement, low cost and short rearing times are advantageous for experiments on retinal diseases. In all animals, the retinal thickness was different in different area. Thus, to track changes in retinal thickness among individual animals, it is critical that measurements are taken at the same location.
Interestingly, we also found the fluorescence in the retinal vessels during FFA was disturbed by the choroid in albino strains, due to the lack of pigment. Therefore, if researchers choose the rats or mice for retinal vessels diseases, they should choose the pigmented strains such as Long Evans rats or C57BL/j mice.
Acknowledgments
Funding: This study was funded by Science and Technology Projects of Guangdong Province (Nos. 2019A030317002, 2017A030303013, 2013B060300003). Funders provided most of the money for this research and participated in research design at the beginning.
Footnote
Reporting Checklist: The authors have completed the ARRIVE reporting checklist. Available at https://aes.amegroups.com/article/view/10.21037/aes-22-54/rc
Data Sharing Statement: Available at https://aes.amegroups.com/article/view/10.21037/aes-22-54/dss
Conflicts of Interest: All authors have completed the ICMJE uniform disclosure form (available at https://aes.amegroups.com/article/view/10.21037/aes-22-54/coif). The authors have no conflicts of interest to declare.
Ethical Statement: The authors are accountable for all aspects of the work in ensuring that questions related to the accuracy or integrity of any part of the work are appropriately investigated and resolved. All animal procedures were performed in accordance with the Association for Research in Vision and Ophthalmology (ARVO) Statement for the Use of Animals in Ophthalmic and Vision Research and approved by the Institutional Animal Ethics Committee of Zhongshan Ophthalmic Center, Sun Yat-sen University (Animal Welfare Assurance No. 2016-120).
Open Access Statement: This is an Open Access article distributed in accordance with the Creative Commons Attribution-NonCommercial-NoDerivs 4.0 International License (CC BY-NC-ND 4.0), which permits the non-commercial replication and distribution of the article with the strict proviso that no changes or edits are made and the original work is properly cited (including links to both the formal publication through the relevant DOI and the license). See: https://creativecommons.org/licenses/by-nc-nd/4.0/.
References
- Szabelska A, Tatara MR, Krupski W. Morphological, densitometric and mechanical properties of mandible in 5-month-old Polish Merino sheep. BMC Vet Res 2017;13:12. [Crossref] [PubMed]
- Garabedian C, Champion C, Servan-Schreiber E, et al. A new analysis of heart rate variability in the assessment of fetal parasympathetic activity: An experimental study in a fetal sheep model. PLoS One 2017;12:e0180653. [Crossref] [PubMed]
- Chatterjee M, Faot F, Correa C, et al. A robust methodology for the quantitative assessment of the rat jawbone microstructure. Int J Oral Sci 2017;9:87-94. [Crossref] [PubMed]
- Di Nardo F, Anfossi L, Ozella L, et al. Validation of a qualitative immunochromatographic test for the noninvasive assessment of stress in dogs. J Chromatogr B Analyt Technol Biomed Life Sci 2016;1028:192-8. [Crossref] [PubMed]
- Cowin SC, Hart RT, Balser JR, et al. Functional adaptation in long bones: establishing in vivo values for surface remodeling rate coefficients. J Biomech 1985;18:665-84. [Crossref] [PubMed]
- Alexander A, Sherman J, Horn D. Fundus fluorescein angiography: a summary of theoretical concepts and clinical applications. J Am Optom Assoc 1979;50:53-63. [PubMed]
- Huang D, Swanson EA, Lin CP, et al. Optical coherence tomography. Science 1991;254:1178-81. [Crossref] [PubMed]
- Anger EM, Unterhuber A, Hermann B, et al. Ultrahigh resolution optical coherence tomography of the monkey fovea. Identification of retinal sublayers by correlation with semithin histology sections. Exp Eye Res 2004;78:1117-25. [Crossref] [PubMed]
- Rosolen SG, Rivière ML, Lavillegrand S, et al. Use of a combined slit-lamp SD-OCT to obtain anterior and posterior segment images in selected animal species. Vet Ophthalmol 2012;15:105-15. [Crossref] [PubMed]
- Strouthidis NG, Grimm J, Williams GA, et al. A comparison of optic nerve head morphology viewed by spectral domain optical coherence tomography and by serial histology. Invest Ophthalmol Vis Sci 2010;51:1464-74. [Crossref] [PubMed]
- Alkin Z, Kashani AH, López-Jaime GR, et al. Quantitative analysis of retinal structures using spectral domain optical coherence tomography in normal rabbits. Curr Eye Res 2013;38:299-304. [Crossref] [PubMed]
- Bartuma H, Petrus-Reurer S, Aronsson M, et al. In Vivo Imaging of Subretinal Bleb-Induced Outer Retinal Degeneration in the Rabbit. Invest Ophthalmol Vis Sci 2015;56:2423-30. [Crossref] [PubMed]
- Koinzer S, Bajorat S, Hesse C, et al. Calibration of histological retina specimens after fixation in Margo's solution and paraffin embedding to in-vivo dimensions, using photography and optical coherence tomography. Graefes Arch Clin Exp Ophthalmol 2014;252:145-53. [Crossref] [PubMed]
- Ruggeri M, Wehbe H, Jiao S, et al. In vivo three-dimensional high-resolution imaging of rodent retina with spectral-domain optical coherence tomography. Invest Ophthalmol Vis Sci 2007;48:1808-14. [Crossref] [PubMed]
- Yamauchi Y, Yagi H, Usui Y, et al. Biological activity is the likely origin of the intersection between the photoreceptor inner and outer segments of the rat retina as determined by optical coherence tomography. Clin Ophthalmol 2011;5:1649-53. [Crossref] [PubMed]
- Dysli C, Enzmann V, Sznitman R, et al. Quantitative Analysis of Mouse Retinal Layers Using Automated Segmentation of Spectral Domain Optical Coherence Tomography Images. Transl Vis Sci Technol 2015;4:9. [Crossref] [PubMed]
- Ehinger B, Zucker CL, Bruun A, et al. In vivo staining of oligodendroglia in the rabbit retina. Glia 1994;10:40-8. [Crossref] [PubMed]
- Haddad A, Salazar JJ, Laicine EM, et al. A direct contact between astrocyte and vitreous body is possible in the rabbit eye due to discontinuities in the basement membrane of the retinal inner limiting membrane. Braz J Med Biol Res 2003;36:207-11. [Crossref] [PubMed]
- Jo YH, Sung KR, Shin JW. Effects of Age on Peripapillary and Macular Vessel Density Determined Using Optical Coherence Tomography Angiography in Healthy Eyes. Invest Ophthalmol Vis Sci 2019;60:3492-8. [Crossref] [PubMed]
- Ito Y, Sasaki M, Takahashi H, et al. Quantitative Assessment of the Retina Using OCT and Associations with Cognitive Function. Ophthalmology 2020;127:107-18. [Crossref] [PubMed]
- McLenachan S, Magno AL, Ramos D, et al. Angiography reveals novel features of the retinal vasculature in healthy and diabetic mice. Exp Eye Res 2015;138:6-21. [Crossref] [PubMed]
- Zhao T, Zhang J, Zhang Y, et al. Vascular Endothelial Growth Factor Receptor 2 Antibody, BC001, Attenuates Laser-Induced Choroidal Neovascularization in Rhesus Monkeys (Macaca mulatta). J Ocul Pharmacol Ther 2015;31:611-6. [Crossref] [PubMed]
- Sugimoto Y, Mochizuki H, Miyagi H, et al. Histological findings of uveal capillaries in rabbit eyes after multiple intravitreal injections of bevacizumab. Curr Eye Res 2013;38:487-96. [Crossref] [PubMed]
- Yuan YZ, Yuan F, Xu QY, et al. Effect of Fufang Xueshuantong Capsule on a rat model of retinal vein occlusion. Chin J Integr Med 2011;17:296-301. [Crossref] [PubMed]
- Lin CH, Liao PL, Hsiao G, et al. Long-term Fluorometholone Topical Use Induces Ganglion Cell Damage in Rats Analyzed With Optical Coherence Tomography. Toxicol Sci 2015;147:317-25. [Crossref] [PubMed]
- Ehrenberg M, Ehrenberg S, Schwob O, et al. Murine fundus fluorescein angiography: An alternative approach using a handheld camera. Exp Eye Res 2016;148:74-8. [Crossref] [PubMed]
Cite this article as: Xian B, Zhao M, Peng Y, Wang W, Li Z, Zhang H, Li W, Huang B. Fundus photography, fundus fluorescein angiography, and optical coherence tomography of healthy cynomolgus monkey, New Zealand rabbit, Sprague Dawley rat, and BALB/c mouse retinas. Ann Eye Sci 2023;8:10.